Accessibility disclaimer: To obtain information contained in document files on this page in an accessible format please contact the Electron Microscopy Center at (812) 856-1457 or through email at dagmorga@indiana.edu
Radiation Damage
Although the materials science electron microscopy community divides the world into "biological or soft" and "non-biological or hard" materials (where non-biological/hard materials are essentially the things that are resistant to the electron beam), electrons are ionizing radiation and everything will damage if exposed to an electron beam for a long enough time. This "radiation damage" can be quite easy to see (unstained protein molecules visibly damage very quickly, some zeolites are quite sensitive to the beam, certain metallic nano-particles melt in the electron beam, etc.) or it may require prolonged exposure to the electron beam to see any sort of damage. In any case, it is very important to remember both that everything will eventually damage in the electron beam and (perhaps more importantly) that if one does not deliberately look for radiation damage effects, subtle damage may simply be missed.
There is a lot of talk (especially in the cryoEM community) about the general topic of radiation damage (more correctly, electron beam induced radiation damage), but what is often missing is any sense of the scale of the damage or even the type of damage that happens. The general consensus is that atomic structure in all biological and even in many materials science specimens is wrecked by exposure to the electron beam, but here are some ideas and numbers (thanks originally to Reuben Diaz) that will help bring this concept of damage into context:
- a typical, high-dose EM image might be recorded using as much as 200 electrons / Å2 (or more)
- 1 Coulomb = 6.242 × 1018 electrons
- from radiology, 1 rad is the radiation dose causing the absorption of 100 erg / g and for carbonaceous material, 1 Coulomb / m2 of 100kV electrons equates to ~4 x 107 rads
- 1 Coulomb / m2 is ~6 electrons / nm2 or ~0.06 electrons / Å2
- 200 electrons / Å2 would be ~3333 Coulombs / m2, or ~1.2 x 1011 rad
- and finally, 106 rad is enough to kill anything alive (except Deinococcus radiodurans and comparable radiation resistant bacteria) and to deactivate all enzymes!
Another way to think about this is more visual:
- a typical, low-dose EM image might be recorded
- using on the order of 10 electrons / Å2 using the numbers from above, 10 electrons / Å2 is a equivalent to a radiation exposure of ~6.6 x 109 rad
- which is about the same exposure as standing 30 m from a 10 megaton atomic explosion!
All of which means that the scale of the damage due to the electron beam is enormous.
Biological materials (comprised mostly of elements with low atomic number) show visible damage quite easily in the electron beam, and bond breakage (including carbon-carbon bonds) is the major event that occurs. Almost everyone who has done cryoTEM has seen bubbles form in the vitreous ice matrix around a biological specimen and in indeed, the bubbles are hydrogen gas at very high pressure (in excess of 1000 atm). This hydrogen gas appears to form via complex reactions that involve radical formation from not only water but also the organic molecules. This conclusion is based on the observation that pure water does not bubble until the electron dose necessary to bubble protein solutions has been massively exceeded, giving the organic molecules some sort of significant role in this process. Anecdotal evidence also indicates that some compounds bubble more readily than others. For example, many people avoid glycerol in frozen, hydrated specimens because of the tendency to bubble faster than similar frozen solutions that do not contain glycerol. There are also reports that Escherichia coli bacteria bubble more readily than many other bacterial strains.
The bubbling shown in the images below is an indication of severe radiation damage. Bubbles tend to occur first on "surfaces" such as the lacy carbon film shown in these images, and is likely to be the result of the carbon layer acting as a diffusion barrier to hydrogen molecules formed via radiolysis. This "surface effect" can be seen at edges of isolated macromolecular assemblies, where there is also a (weak) barrier to diffusion and a source of non-water-based hydrogen radicals.
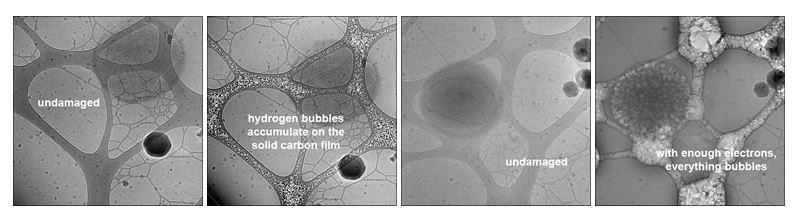
In the field of structural biology, bond breakage followed by structural reorganization can be observed and measured in biological materials, and such damage/disorder can be show to happen in crystalline samples well before visible bubbling appears. Several studies based on protein crystals have shown that higher resolution order disappears more rapidly than lower resolution order both at liquid nitrogen (-195.8° C or 77° K) and liquid helium (-269° C or 4° K) temperatures, that the onset of measurable damage occurs with a dose of a few electrons per Å2 and that lowering the temperature of the specimen provides some degree of tolerance to these effects.
- Cryoprotection in Electron Microscopy from the Journal of Microscopy
- Electron Radiation Damage to Protein Crystals of Bacteriorhodopsin at Different Temperatures from ScienceDirect
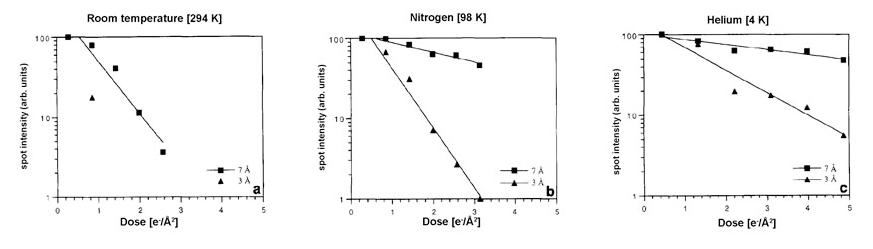
For example, the amount of disorder caused by the same electron dose is reduced by at least a factor of five-fold when the specimen temperature is lowered from room temperature to liquid nitrogen temperature, and by at least another two-fold when the temperature is further lowered to that of liquid helium. Since bond breakage by the ionizing electron beam is unlikely to be affected by temperature, these protective effects are most likely the result of changes in the structural reorganization process itself (i.e., processes like thermal vibration and diffusion are expected to be functions of temperature, and these could easily affect the way broken bonds reorganize in the damaged materials).
These and similar studies have produced the general rule that low dose imaging (minimal dose imaging) of frozen, hydrated biological samples should limit the electron dose in a single image to about 10 electrons Å2 (bearing in mind that while the damage caused by this electron dose is not zero, it is tolerable). This limit is rather flexible and is really a function of the desired resolution and the detailed behaviour of a particular specimen. For example, if one is only attempting to retain 20-25 Å resolution information in images or a three-dimensional (3d) reconstruction, a significantly higher electron dose is permissible. On the other hand, if the goal is near-atomic resolution, then the dose should be as low as can be used while maintaining the ability to do any necessary image processing (e.g., particle selection and/or defocus determination). Recent changes in technology such as direct electron detecting cameras have made it much easier to record low dose images without compromising steps necessary for further image processing.
It is also important to remember that the radiation damage studies have shown that crystals from different types of protein damage at different rates and that even different crystallographic reflections within a given crystal can damage at different rates. This raises the very real possibility that protein crystals are not necessarily a good model system for the effects of radiation damage on other biological specimens such as helical proteins assemblies, viruses or isolated macromolecular complexes (so-called "single particles") and that nucleic acid-containing specimens may respond somewhat differently to the electron beam. Some studies have examined the effects on the resulting 3d reconstruction of increasing the electron dose for images of single particles. These effects are harder to quantify (for various technical reasons not discussed here) than the radiation damage studies of protein crystals mentioned above, but the overall conclusions are the same: resolution decreases in a dose dependent manner, higher resolution information vanishes faster than lower resolution information and different areas of a single particle (or different spacings in a protein crystal) appear to damage at different rates.
There is also beginning to be a consensus that soft, non-biological materials exist (i.e., non-biological materials that damage in the electron beam) and that the materials science community needs to address radiation damage issues directly. One example of inorganic materials that damage in the electron beam has been mentioned above: numerous studies have shown that zeolites damage in the electron beam, albeit at doses and rates significantly slower than biological materials.